Nuclear Magnetic Resonance is a powerful tool for studying proteins. Among the most complex of macromolecules, they can be made up of hundreds or even thousands of amino acids. Biologists who study them want to know not only what atoms make up these molecules, but how these atoms are arranged. Mapping proteins requires time, patience, expertise and sensitive instruments such as the MagLab's 900 MHz NMR magnet.
Making this job even more difficult is the fact that proteins can't tolerate heat: Even a little bit will essentially cook them, ruining an experiment. For years biologists worked as best they could under these constraints. Until, that is, MagLab probe designer Peter Gor'kov came up with a solution — by accident.
One night in 2004, Gor'kov was trying to wash off the day's frustrations under a hot shower. Some "users," as visiting scientists are called around here, needed to irradiate samples with a stronger electromagnetic field to obtain better data on the proteins they were studying. Gor'kov had sunk a lot of time and money into designing and building a probe that would give it to them. But the users insisted that, despite the extra electric power going into it, the field wasn't getting any stronger, and their data wasn't getting any better.
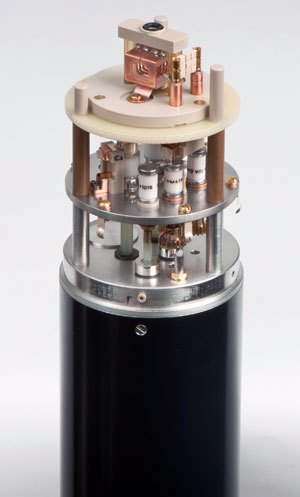
Probe head for the low-E probe.
"I was very upset that evening," recalls Gor'kov, "because it just went on and on and on, and the users complained and complained and complained. We spent already two years on this project, trying to make the biochemists happy."
NMR probes are equipped with radio frequency (RF) coils; the sample that's being studied sits inside an RF coil, or coils. When electricity is sent through a coil, it generates an electromagnetic field in the sample at a carefully selected radio frequency. Nuclei of each atom in the sample respond to one particular frequency, sending back signals that give scientists information on the composition of the sample and the arrangement of its atoms.
Gor'kov had tried tweaking the probe in various ways so that it would deliver stronger electromagnetic field and better data could be gleaned. But to no avail. His bosses were urging him to throw in the towel.
"I tried every approach I knew of," Gor'kov says. "I thought, 'There's nothing more left — I can only try one more thing, and then that's it.'" If it didn't work, he decided, he would grudgingly concede defeat.
It was in the shower that Gor'kov decided the problem lay in how the RF coils in the probe were arranged. RF coils irradiate samples at two different frequencies. The lower of these two frequencies targets the atoms — nitrogen or carbon, for example — which the scientist is trying to map. The higher frequency interacts with the sample's hydrogen atoms in a way that keeps them from interfering with the target data. (This common technique, called proton decoupling, yields clearer results.) In conventional single-coil arrangements, the respective magnetic fields generated by the two frequencies were colinear. In such an arrangement, the probe must include components that isolate the parts of the circuitry operating at different frequencies. Gor'kov suspected that these isolation components were siphoning off energy intended to create a stronger electromagnetic field.
So he built a probe with two RF coils arranged perpendicular to each other, eliminating the crosstalk occurring between two parts of the circuit.
Much to Gor'kov's relief, the modified probe worked — and how. His stroke of genius solved not one problem, but two. As it turned out, the new coil arrangement produced relatively little heat, protecting the sample positioned inside it from death by frying.
And so it was that, without even trying, Gor'kov solved an intransigent problem in solid-state NMR spectroscopy. Gor'kov could not believe he hadn't thought of it sooner: It was one of those moments when the base of the hand flies up to the forehead, producing a dull thump and a self-flagellating "Duh!"
"It's so simple," says Gor'kov, with the benefit of hindsight. "If we had started approaching the problem in the right way, we would have solved it immediately."
The breakthrough was significant. Gor'kov's new "low-E" probe (E as in electrical field) reduced the amount of heat produced in the sample tenfold. This made it a better choice for proteins and other samples high in salt, which readily conducts electricity. The energy delivered to the RF coil quickly dissipates in such samples, heating them up like a microwave, instead of increasing the strength of the electromagnetic field. The low-E design is also simpler than the probes from which it evolved, offers more room for a larger volume of sample and yields a more homogenous magnetic field.
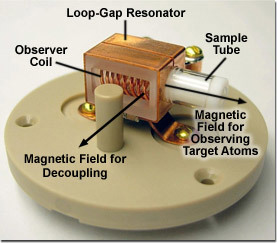
The RF coil of the low-E probe.
As shown in the photo at left, a solenoid coil of several turns is situated inside a second, box-like coil made of a single loop of copper. The inner solenoid is the "observer coil," emitting the radio frequency of the target nuclei. The outer coil irradiates the sample at the frequency of decoupling. The coils generate alternating magnetic fields that run perpendicular to each other, avoiding crosstalk. The round window in the outer coil allows scientists to slide their samples inside the inner coil. Bruker Biospin Corp. has taken this clever design and built a low-E probe that is now commercially available.
It was the choice of the outer coil, known as a loop-gap resonator (LGR), that saved the samples from destruction by heat. Gor'kov borrowed the idea of using this device from the field of Electron Magnetic Resonance (EMR), another spectroscopy tool used here at the MagLab. The interdisciplinary environment of the lab, where scientists from different fields come to use a wide variety of tools and techniques, fosters the kind of creative thinking that inspired Gor'kov to find an EMR solution to an NMR problem.
"EMR scientists used loop-gap resonators for their applications since I was in elementary school," says Gor'kov. "In the MagLab, you can tap into that knowledge while waiting on coffee in the break room."
Story by Kristen Coyne
For more information, please contact Public Affairs.