This isn't a story about a grand discovery or life-changing eureka moment.
Get real: Scientists get precious few of those. Most of the time, they're wading through the salty, smelly perspiration that makes up 99% of what they do.
Well, maybe not 99%. Maybe that ratio is more like 1% inspiration, 95% perspiration and 4% incremental breakthroughs.
This story is about one such mini milestone. One of those little triumphs that motivates you to keep trudging down the grueling, boring stretches. It's not a eureka moment, not even an "Aha!" moment. It's more like a "Phew!" moment, a reassurance you're slogging up the right path. It's just enough to keep you keeping on.
If you're picturing a lone scientist schlepping valiantly uphill, imagine this instead: A whole squad of them. Science is, after all, a team sport.
Every good team has a captain, and the one in this story is no exception. Meet Shanti Deemyad captain of Team Lithium.
The incredible lightness of lithium
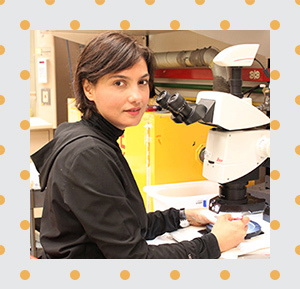
Shanti Deemyad
Photo credit: Saveez Saffarian
Deemyad is everything you want in a captain. She's smart, enthusiastic and super positive. An associate professor of physics at the University of Utah, she's a former recipient of the prestigious National Science Foundation Early Career Award. She specializes in high-pressure physics, and specializes even further in the element lithium.
Used widely in everything from batteries to medicine, lithium is highly reactive and has to be stored in mineral oil to prevent it from interacting with neighboring atoms and molecules. It's also the third lightest element on the periodic table (after hydrogen and helium) and (under normal conditions) the lightest of all the solids and metals.
That's what draws Deemyad to lithium. For someone interested in probing the subatomic realm, the lighter the element, the better. Lighter, Deemyad explains, means more quantum: As you subject atoms of light elements to weird experimental conditions, they are more likely to do curious things, like transform into a different phase of matter or quantum state.
"In stuff that is very light, each particle behaves more like a wave," Deemyad explains. "Everything is more unstable."
Deemyad, petite and with a ready smile, wants to make lithium even more unstable to unlock more quantum magic. So she compresses it. Pressurizing materials is one trick scientists use to tease out interesting behaviors like superconductivity — 100% efficient electricity in which current-carrying electrons encounter zero friction.
Under pressure, Deemyad says, "Everything starts changing. The homogeneity of everything goes away."
The big squeeze
The more you compress, the more you can discover. In her own lab, Deemyad is able to put samples under pressures as high as what exists at Earth's core. But working under pressure while in high magnetic fields is a very different challenge. For that, Deemyad needs to come to the MagLab, which has the expertise and equipment for those experiments. MagLab scientists have developed tiny, specialized pressure cells that tolerate high magnetic fields and fit into the small space inside the magnets; they have also developed sophisticated techniques to measure the signals they are looking for under those extreme conditions.
"It would not be feasible to develop those techniques myself," Deemyad says.
MagLab physicists (left to right) Stan Tozer, Audrey Grockowiak and William Coniglio collaborated with Deemyad's group on her MagLab research probing the mysteries of lithium.
Photo: Stephen Bilenky
With a team comprising her students, MagLab specialists and a theory group led by Neil Ashcroft and Roald Hoffmann of Cornell University, Deemyad has been experimenting on lithium at the MagLab for more than five years. They have labored through the science grunt work, gradually reaching higher pressures in the challenging environment of a high-field magnet. Last year, the group hit a mini milestone, successfully creating pressures of 1 gigapascal (GPa) and observing promising data. (As a comparison, 0.5 GPa is the pressure at 5,000 meters below the sea.1) It wasn't the holy grail. It wasn’t science Shangri-La. But it was an affirmation they were on the right track and enough to earn them access to a stronger MagLab magnet for future experiments.
The amazing disappearing sample
So, one of the great things about lithium, from a science perspective, is it's light.
But one of the worst things about lithium, from a technical perspective, is ... well, that it's light.
Lithium has caused Audrey Grockowiak no end of grief. Grockowiak is a physicist in the research group of Stan Tozer at the National MagLab, which specializes in high-pressure physics. That means she spends a lot of time at the microscope designing, building and loading tiny pressure cells, which compress samples between two diamonds. It's a tricky technique with the easiest of samples. Finagling lithium in a pressure cell, however, is exceptionally vexing.
"You try to put this in your pressure cell and — pffft — there it goes. It floats away," Grockowiak says. "It's really a pain."
Audrey Grockowiak holds a tiny pressure cell, which compresses the sample before it enters a magnet.
Photo: Stephen Bilenky
And that's just one hurdle. The team has also struggled with how to distribute the pressure from the two diamond tips evenly around the entire lithium sample, which is very soft at high pressures. They tried coating the sample in mineral oil but, after months of trial and error, the team still wasn’t sure that was the right technique.
If exposed to air, lithium degrades within seconds and its nice, metallic shine turns dark and dull. So while cutting the sample to the right size (just 40 microns thick, roughly the width of a human hair), Grockowiak had to keep it in oil on the microscope slide, then transfer it into the pressure cell as fast as possible.
"Those samples are the most challenging I've had to work with," Grockowiak says.
Physicist William Coniglio faced his own challenges on the experiments. His job was to connect Grockowiak's tiny pressure cell to another teensy device (called a tunnel diode oscillator) so they could run electricity through the lithium and measure the resistance.
"Sometimes you get lucky and you have a really good day, and sometimes you get unlucky and things are misbehaving," explains Coniglio. "And then sometimes, even if you are having a good day, the sample decides how good it is. There are all these factors that are out of your control."
What are electrons trying to tell us?
All of which begs the question: Why go to all that trouble?
Team Captain Deemyad knows: In science as in sports, no pain, no gain.
Imagine an astronomer obsessed with the idea of life on other planets. She spends countless nights at the observatory operating her telescope, endless days mining the data for the good bits, hoping for a signal from deep in the universe, something whispering, "Yoo-hoo! Over here!"
Deemyad is straining to hear little signals, too, but hers are from electrons in lithium — the lightest subatomic particles in one of the lightest atoms. These signals are called quantum oscillations, which are wavelike undulations measured in the electrical resistance of the electrons. Scientists use them to generate a kind of map of the material known as the Fermi surface, which can only be measured using high magnetic fields. The Fermi surface is a kind of Rosetta Stone for the material, just waiting to be decoded, containing a motherlode of information about how its active electrons behave and interact.
"Seeing the quantum oscillations gives us a lot of information about both the electronic and crystal structures of lithium that is otherwise hard to understand," Deemyad said.
Ultimately, physicists like Deemyad are looking for the underlying principles behind the behaviors they create in extreme conditions, knowledge that could one day help scientists design new materials that exhibit those same behaviors under the normal conditions in which we all live.
A bad week ends on a high-field note
Typically, scientists who come to the MagLab use a magnet for a week. After inserting their small sample into the middle of the magnet coil, they tweak different parameters — the magnetic field, pressure and temperature around their sample — then compare how it behaves under varying conditions.
One Friday evening in February, Tushar Bhowmick, a graduate student in Deemyad's group, was at the end of an exhausting week at the MagLab. Because Deemyad had other obligations, Bhowmick was working without his advisor, alongside the MagLab team. Earlier that week, they had had some successes — they got a pressure cell to operate at 1.7 GPa and observed quantum oscillations — as well as some setbacks. One day they struggled with a bad pressure cell, ending their shift with nothing to show for it. Then a freak storm closed all of Florida State University, where the MagLab is headquartered: Another day with no data.
Tushar Bhowmick observes data coming in at the MagLab.
Late that Friday, in his last hours with the magnet, Bhowmick was analyzing the most recent data from a lithium sample under a whopping 2.8 GPa of pressure in magnetic fields of 33 to 35 tesla x Tesla, or T, is a unit of magnetic field strength; a strong refrigerator magnet is .01 tesla, and a typical MRI machine is 1.5 to 3 tesla. The MagLab's strongest persistent magnet has a field of 45 teslas. . He finally saw what may have been the signal they'd been waiting for — a peak in the data that suggested quantum oscillations. But he needed to try again: Some extraneous information, called noise, made the data unclear. So he asked for another 33-to-35 tesla run, hoping for a stronger signal.
And he got it: Not only did he see another peak, but it was much more distinct than the previous one.
Data from Deemyad's lithium experiments. The purple peak shows the team's most recent results: Quantum oscillations at 2.8 GPa of pressure.
"I saw a very clear peak, and I realized we have quantum oscillations," Bhowmick says. "That was an enormous joy for me."
Bhowmick threw his arms up in victory; Grockowiak captured his elation on her cell phone, making the image you saw at the top of this story .
She was beaming too.
"My efforts are paying off," she says. "I didn't spend all of those hours and days and nights at the microscope for nothing."
- Listen to Deemyad explain her research in this 4-minute video
- Visit her research group’s website to learn more
Meanwhile, holding down the fort back in Utah, Deemyad wasn't expecting good news. Because they had struggled with the pressure medium so long, she suspected it might be time to throw in that towel and try a different one — probably helium. That would take them back to square one in technique development.
Deemyad was following the experiment closely when she suddenly got a text from Grockowiak: It read, "QO at 28.5 kbar" (translation: quantum oscillations at 28.5 kilobars). She attached the photo of Bhowmick.
"I couldn't believe it," Deemyad says. "I was just super excited."
"These results are not only exciting on their own, but they are also proof of principle that we can do way more," Deemyad continues. "The door opened up to doing a whole new level of experiment."
The success gave Deemyad confidence that her team will be able to get data from lithium up to 20 GPa. At those pressures, she says, they will be able to observe how electrons behave just before they transition into a superconducting state.
"Being able to get here changes the whole story," Deemyad says.
And that's where this particular story ends … at least until the next "Phew!" moment.
Other scientists contributing to this research include Stanimir Bonev from Lawrence Livermore National Laboratory and Sabri Elatresh from Cornell.
Story by Kristen Coyne