In early 2006, like a stork with a special delivery, a space capsule from NASA’s storied Stardust mission drifted down to the Utah desert beneath a white and orange parachute. While there were no swaddled babies on board, the capsule did contain a precious payload related to birth – not human, but cosmic. Cushioned in a special gel was a smattering of comet dust originating from the outer limits of the solar system.
The Stardust treasure trove contained the first extraterrestrial material imported to the planet since the Apollo astronauts hauled back moon rocks decades earlier. Though weighing a fraction of an ounce, the comet dust promised to unlock even more tantalizing secrets than those lunar artifacts: The particles came from much farther away in the solar system, and dated from a time before the moon – or even the planets – existed.
During the $212-million Stardust project, the spacecraft traveled more than 3 billion miles over seven years, rendezvous-ing with the comet Wild 2 during the second of three orbits around the sun. The end of the mission marked the beginning of another adventure: Examining the comet particles with powerful scientific instruments called mass spectrometers, which are able to identify what isotopes the stuff is made of.
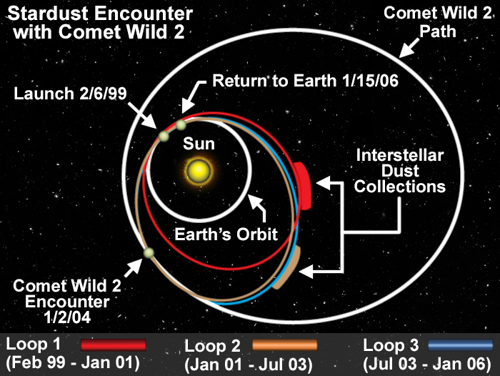
Isotopes are atoms of the same element that have different atomic weights, due to varying numbers of neutrons (the neutrally-charged particles found in an atom's nucleus). Depending on the isotopes that make up a particular sample, researchers glean clues about its origin and how it was formed.
Physics Factoid
All elements have stable isotopes; some have more, some less. Hydrogen, for example, has only two, while xenon has nine.
Research on Stardust comet dust is underway in labs worldwide, including within the Geochemistry Program at the MagLab. Though less sexy than a rocket ship blasting through space at 48,000 miles per hour, this phase of the project is just as significant and compelling. It takes place, however, on an entirely different scale, featuring numbers not only mind-bogglingly large, but mind-bogglingly small. The particles scientists will be studying are measured in microns (µm) – even fractions of microns. A human hair, with a width of about 100 microns, is vast compared to most of the comet particles researchers will be slicing, dicing and scrutinizing.

But good things come in small packages, and these tiny particles may hold answers to some of our weightiest questions. How did comets form? Did material from outside our solar system contribute to their makeup? Exactly what building blocks were used to create our solar system? Did comets make life on Earth possible by transporting critical elements to the planet? Could they do the same for other planets?
Munir Humayun, an associate professor in geochemistry at Florida State University working at the MagLab, is among the elite group of scientists granted access to the Stardust booty. He even designed a special type of mass spectrometer to measure the particles and reveal data that could shine a light on how they and the solar system were formed.
Journey Back in Time
Comets are so fascinating to scientists because they contain the stuff with which the solar system was created. While other celestial bodies have evolved over time, chemically and physically, due to radioactive heat and collisions with each other, comets are considered comparatively pristine. Scientists estimate that trillions of them populate our solar system. Though a tiny minority of them venture closer to us, they mostly keep to themselves on the frigid periphery called the Oort Cloud (well beyond Pluto) and have been preserved there in roughly the same way pork chops keep in your freezer.
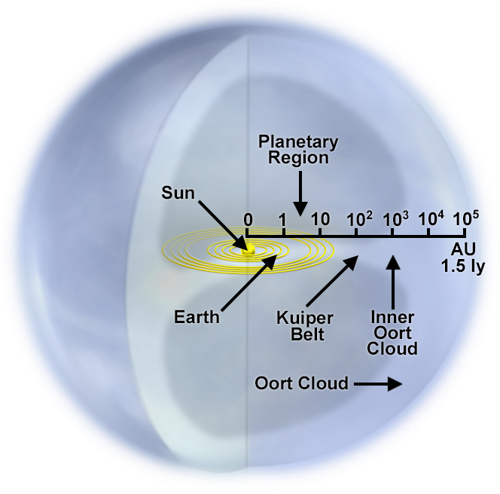
Each grain is a tiny time capsule bursting with data. If these comets contain the building blocks of our solar system, they must then contain the building blocks of the Earth and, it would follow, of all life on the planet. These comets are, in other words, our atomic ancestors.
Physics Factoid
The highly elliptical orbits of comets sometimes bring them near large planets. These close encounters can alter their orbits, sometimes drawing comets closer to the Earth and sun (as happened to Wild 2 in 1974 after passing near Jupiter), sometimes ejecting them farther out.
Some 4.6 billion years ago our solar system – at that time an immense cloud of gas and dust – began to form. Catalyzed by some outside galactic event – perhaps a shockwave from a supernova – this nebula began to collapse under its own gravity, heating up and compressing in the process. At the center formed what would become our sun; the gas and dust that orbited it eventually coalesced into planets. Much of the matter entering the inner solar nebula may have been vaporized by the heat, erasing all knowledge of its past.
Well, not all of it. After the sun and planets formed, some material was left over on the fringe of the new solar system, sort of like the dusting of unused flour bordering your countertop after you roll out your pastry dough and pop it in the pie plate. This material was scraped together and the solar system’s fleet of comets was born. So scientists believe.
Wild 2 and other comets contain this ur-matter, these unadulterated ingredients that went into our star and planets before they got baked. That’s why scientists salivate at the thought of what comet dust could tell us.
Parsing Particles
One of the problems scientists face in their analysis of Stardust samples is the sub-microscopic scale at which their work must be conducted. At the MagLab, FSU’s Humayun responded to this challenge with his innovative design for a mass spectrometer.
Physics Factoid
Scientists use a seemingly endless variety of mass spectrometers, depending on their research needs. To name just a few:
- Tandem Mass Spectrometer (MS/MS)
- Gas chromatography-mass spectrometer (GC/MS)
- Fourier Transform Ion Cyclotron Resonance Mass Spectrometer (FT-ICR MS)
- Time-of-Flight Mass Spectrometer (TOF MS)
- Electron Spray Ionization Mass Spectrometer (ESI MS)
- Quadropole Ion Trap Mass Spectrometer(QIT MS)
Mass spectrometers, in a nutshell, are machines that give scientists a look at the composition and origin of a material by analyzing and quantifying its atoms and molecules. They do this by vaporizing the sample (if it’s not already a gas) to make it easier to work with, ionizing it to give it a charge, then seeing how those ions react to a magnetic field. That reaction tells scientists how much each particle weighs – or, more precisely, what its mass is. The machine then sorts and counts these particles, revealing the specimen’s chemical makeup. The machine is able to distinguish among isotopes. Scientists can learn a lot from knowing how many of which isotopes make up a certain sample.
Spectrometers have served scientists well ever since they were invented a century ago. A number of designs are now in use (the Stardust spaceship even had one on board), with applications that include detecting steroid use in athletes and locating oil deposits. When NASA solicited ideas for better ways to analyze their comet dust, Humayun proposed a novel twist on an existing type of spectrometer called a Laser Ablation Inductively Coupled Plasma Mass Spectrometer (a mighty mouthful that can be shortened into LA-ICP-MS).
To understand the amount of data this first-of-its-kind machine will be able to extract from the comet particles, we need first to understand the basic principles of mass spectrometry – MS for short.
Ladies and gentlemen, start your brain cells!
Science in the Fast Lane
Got those neurons revved up? Excellent. Here’s what a traditional mass spectrometer looks like.

Mass spectrometers are really not so hard to understand; it’s just that some people seem to find them intrinsically less interesting than spaceships. Yet beneath their invisible-to-the-naked-eye microanalysis lies a drama every bit as compelling as a NASCAR race.
Let’s imagine you’ve nabbed a front-row seat at the Indianapolis 500. It’s been an exciting and exceptionally close race, and now three cars are in the last lap, careening toward the final curve.
Now, imagine that those 3,400-pound speed demons are atoms, and you’ll begin to understand what happens in a mass spectrometer.
The core of most MS machines is a magnet, placed outside a curving tube through which the sample passes. Its field disrupts (and in so doing, helps to measure) the steady stream of atoms passing by. Of course, race cars are subject to different laws of physics as they speed around a bend, but you get the idea – or you will in a minute. (These particles, by the way, actually zoom past the magnet at speeds Jeff Gordon could only dream of, measurable in nanoseconds.)
Before we see how this atomic race ends up, let’s shift into reverse and return to the starting line to get a clearer picture of what’s happening.
Physics Factoid
Mass spectrometers have lots of industrial and research applications. In biotechnology they’re used to analyze proteins and peptides, pharmaceutical companies use them to develop new drugs and doctors use them for neonatal screening. Also, geologists study oil composition with them while environmental scientists analyze water and food samples for contamination … in addition to many other applications.
Mr. Gordon never won a race on foot. Likewise, any substance that’s going to make it from one end of an MS to the other needs a means to get there. That happens through vaporization. Turning a solid or liquid sample into a gas gives it the properties it will need to travel through the machine.
Now that we’ve got a means of getting from point A to point B, we’ll need some juice. To fuel the vaporized atoms, which are electrically neutral, the mass spectrometer turns them into ions. There are several ways of going about the ionization process. In this example, let’s bombard them with a stream of electrons, which in turn knock the electrons circling the specimen atoms out of their orbits. The loss of a negatively-charged electron leaves the atom with a positive charge, creating an ion that will respond to the field of the magnet it is about to encounter.
OK. So far we’ve got the driver (the atom), the car (the vaporization) and the gasoline (ionization). To burn rubber, we’ll also need to depress the accelerator. As it happens, the mass spectrometer features a chamber called the accelerator, in which electrical currents are used to give the ions a starting push. Each is given the same kinetic energy, making this a very fair race. This whole process, by the way, takes place inside a vacuum, eliminating potentially meddlesome air molecules that would otherwise interfere with the flow of the ions. After all, NASCAR drivers don’t need to contend with city traffic!
Now buckle your seat belts as we fast-forward in our contest, approaching the final curve. This is where things get really exciting.
Race to the Finish
From the accelerator, the daredevil ions pass into the part of the machine called the mass analyzer, or deflector – so named because the positively charged ions inside will be deflected from their straight paths by a sideways magnetic field. But precisely how much each of the particles is deflected depends upon its mass. (Technically, it is the particle’s mass-to-charge ratio that accounts for its reaction to the magnetic field. It is possible for atoms to lose more than one electron during ionization, but most lose just one electron, and therefore have a charge of 1+. So we’ll dispense with “mass-to-charge ratios” and just call it the mass, for the purposes of this explanation.)
The greater the particle’s mass, the more it will resist the pull of the magnet. Think of how one-and-a-half tons of metal on wheels is able to hug a curve at 150 miles per hour, whereas you in your hatchback would be toast. Take a look at the interactive tutorial below.
In this tutorial, the ions of different colors signify ions of different weights. The blue are the lightest, the green are heavier, and the red are heaviest. Adjust the Magnetic Field Strength and see the effect. In order to make it through the curved tunnel of the mass analyzer, the ions need to have just the right mass, proportional to the pull of the magnetic field (which, in the tutorial, follows the same direction as your eyes as they read these words – straight into the computer screen). If the ions are too light, or too heavy, they will, like an ill-fated race car driver, veer into the inner or outer wall of the deflector tunnel, never crossing the finish line into the attached ion detector, never hearing the crowds roar. But if they have just the right mass, proportionate to the magnetic field, to allow them to travel through the mass analyzer unscathed, the particles will reach the detector and the checkered flags will wave. The arrival of each ion creates a pulse of electrons (kind of like the arrival of a race car creates a roar of applause) and this pulse is recorded.
Physics Factoid
The way ions behave in a mass analyzer (the lighter ions deflecting farther than the heavy ones) is explained by the second of Newton’s laws of motion: “The rate of change of the momentum of a body is directly proportional to the net force acting on it, and the direction of the change in momentum takes place in the direction of the net force.”
So, the MS both weighs and counts. The mass of the ions is deduced by the force of the magnetic field required to guide them into the detector. And the number of ions of that given mass is counted as the ions pass into the detector. To count ions of other masses, the electromagnet’s field is rapidly varied under computer control, so that ions of all masses can be sampled in a fraction of a second.
In this way the range of possible masses for the particles is tested, resulting in a spectrum – the mass spectrum – for the substance under study. That spectrum reveals how many isotopes of a given element are to be found in the material. This is known as the isotope’s relative abundance – relative, that is, to the other isotopes found in the sample. Researchers use this data to glean information on the sample’s history and chemistry: That’s their trophy. Take a look at this tutorial for an example of mass spectra of different elements.
After that mental work-out, you need a rest. Let’s make a quick pit stop before moving on from our by now tired NASCAR analogy. Now that you grasp the MS basics, you’re ready to move to the next level – a bit of a twist on the run-of-the-mill MS we’ve just described.
Two Better than One
Ready? Good!
MS machines come in all shapes, sizes and configurations. The simple mass spectrometer we just described is a single sector MS – made with a single magnetic analyzer. A widely used variation of this is the double sector MS. It has two analyzers – one magnetic, one electrostatic.
Physics Factoid
Ions come in two flavors. Cations (positive) and anions (negative). The word "ion" comes from the Greek word "to go" or "goer." According to this etymology, anions go "up," and cations go "down."
Dual sector MS doesn’t use electron bombardment to ionize the sample (which, as just described, gives all the ions the same speed). Instead, it uses other methods (one of which is described in the following section) that result in ions of the same mass traveling at different velocities.
These different speeds give the ions different trajectories: Some will end up in the detector, many won’t. That’s no good! To get them all in the detector (and accurately counted), they must travel the same speed. So after ions are analyzed by mass (with the magnet), they must be analyzed by speed (or kinetic energy) with the electrostatic field as well. This is how it works.
As you can see in the interactive tutorial below, the ions enter the first (magnetic) analyzer, where they are sorted by mass before a select group is waved ahead into the electrostatic analyzer (this arrangement is sometimes flipped, but the same principles are at work). Traveling at different speeds, they disperse as they take the turn in the electrostatic analyzer. At this rate, only a few will make it into the detector cell; the slower ones will crash into the inner wall, the faster ones will crash into the outer wall, and the count will be inaccurate.
Fortunately, this dispersion is corrected as the ions pass smack dab through an electrostatic field; an electrode on the inner wall emits negative charges, while an electrode on the outer wall emits positive charges. The positive ions, naturally, are repelled from the outer wall. To boot, the laws of physics are such that the closer the ion is to that positively charged wall, the more it will feel the repulsion. So all these particles are drawn into the same middle ground – the same velocity – and travel together, triumphantly, into the detector.
If you’ve still got NASCAR on the brain, think of it this way: The electric field is a hill, sloping from positive down to negative. As the “race cars” approach it, the faster cars shoot up and down the slope more rapidly than the slower cars. But as you can see from the tutorial, the slower cars travel a shorter distance from point A to point B. Given the shorter distance and smaller effect from the positive plate, they catch up with the fast ones by the time they get to the slit. Ladies and gentlemen, it’s a dead heat!
You already have two approaches to MS under your belt. Pretty impressive! Now you’re ready to examine the novel type of MS that Humayun uses to try to solve comet conundrums.
Old Idea, Fresh Take
When considering how to analyze comet dust, Humayun knew that of all existing types of mass spectrometers, the LA-ICP-MS was the best starting point. He knew that the “LA” part – the laser ablation – would allow him to better pinpoint the tiny solid particles he wished to examine, while the “ICP” part – the inductively coupled plasma – would offer both high accuracy and the ability to detect a wide range of metals and trace elements (we’ll explain that momentarily).
It’s an impressive machine. But Humayun needed more. He would, after all, have very little dust to work with: He had to use it as efficiently as possible. With this in mind, he conceived of a way to customize the LA-ICP-MS design for his specific purpose. The adaptation, he says, promises dramatic results, measuring 40 times more information in about the same amount of sample.
Let’s take a closer look at this unique hybrid.
First, the laser will let researchers analyze sample sizes even smaller than those measurable by other machines. In fact, the laser Humayun will use will be able to target areas measuring as little as 4 microns across: You’d have to take one of your hairs and slice it into 20 or more equal-sized pieces – lengthwise – to get something so small. This will allow Humayun to examine comet dust in numerous locations across a single grain. Each of those analyses could reveal a different makeup. This approach yields far more information than if one were to sample the grain as a whole. In other words, data-wise, the sum of the grain’s parts is much greater than its whole.
Second, this machine ionizes samples not by bombarding them with electrons, as in the example described earlier, but by injecting them into an extremely hot plasma created from argon gas. (By plasma we mean a highly ionized gas, not blood). The plasma’s intense heat (in the neighborhood of 7000 kelvin, hotter than the surface of our sun) succeeds in ionizing most of the naturally occurring elements in the periodic table, even the ones that resist ionization. By comparison, another type of machine scientists are using on comet particles, called a Secondary Ion Mass Spectrometer, can only ionize and measure one third of the elements.
Mix and Match
This is where Humayun’s innovation comes into play – and how it achieves its incredible efficiency.
The aerosol produced by the laser is split into two separate samples, which are funneled into two separate mass spectrometers. The machine, in essence, bifurcates.
About 10 percent of the sample is diverted into the first of the two mass spectrometers, featuring a magnet designed especially for fast scanning. Because there are always more of the lighter elements floating around than trace elements, the machine requires just that small portion of the sample to figure out how relatively abundant they are. Attached to this analyzer is the same type of ion detector described in the NASCAR example.
Meanwhile, the remaining 90 percent of the sample takes the road more traveled, into a separate analyzer whose more powerful magnet is calibrated to usher the rarer trace elements into a special set of detectors designed for them. In fact, these few-and-far-between heavyweight elements are serviced by eight detectors; it’s called a multi-ion detector.
The whole machine owes its efficiency to its ability to analyze the same sample in different ways simultaneously (as opposed to sequentially, as in other models). Every little bit counts: While some 400 kilograms of moon rocks have been brought to Earth, Stardust’s total booty amounted to less than one dash of the salt shaker. Nothing should go to waste.
Physics Factoid
Preliminary data from scientists suggest Wild 2 particles are a mix of grains from other stars and materials formed in our own solar system.
Humayun’s machine promises many applications, and is likely to be used by chemical oceanographers and other scientists. But first in line are the comet grains.
If these grains could talk, they’d speak volumes. With machines like Humayun’s, scientists now have ways of making them talk. They may tell us about the history of matter in the interstellar medium, an area of gas and dust between stars. They will tell us what kind of minerals and metals they contain, and perhaps about a star they might have come from. They may tell us they contain water only in its frozen form (comets are, after all, largely ice), or also in the form of hydrated silicates. If the later is the case, it could suggest that comets are actually fragments of bigger bodies.
Most scientists don’t think that’s likely. Then again, much of what we presently think about comets is, as Humayun says, “educated guess work – which may be wildly off.” From Christopher Columbus to Lewis and Clark to Apollo 13, explorers have always encountered the unexpected. And appropriately so: Why dole out hundreds of millions of dollars for a space mission if all that comes of it is a smug “I told you so” from scientists?
Thanks to the Magnet Academy's scientific adviser on this article, Munir Humayun, Associate Professor of Geochemistry at Florida State University and Magnet Lab research associate.
By Kristen Coyne